Designer protein assemblies with tunable phase diagrams in living cells
Phase separation in a cool protein system
Lorenzo Rovigatti
Physics Department, Sapienza University of Rome
Nanoseminars 2021 - 2022, Universiteit Utrecht, January 21st 2022
Please stop me if you have any questions
How things happen in an organism?
- Most of the work is done by macromolecules (proteins and nucleic acids)
- The "right" macromolecules need to find each other $\to$ compartmentalisation


Organelles

- Perform specialised tasks
- Well-separated from the rest of the cell
- Membrane-bound
- Maintain their "identity" over time
- Communicate through molecular signals
More organelles?


Droplets $\to$ phase separation?
"Liquid-liquid" phase separation!
A (gas-like) highly-diluted phase coexist with a (liquid-like) dense phase
Tang, Nature Methods
2019
Membraneless organelles
E. Gomes and J. Shorter, J. Mol. Bio. 2018
- Also known as biomolecular condensates
- Liquid-like (they can and do flow, cfr. Brangwynne et al)
- Made of multivalent (intrinsically-disordered) proteins and/or RNA
- Act as reservoirs of biomolecules or as microreactors
- The mechanisms behind their formation are linked to the pathogenesis of several diseases (e.g. Alzheimer's, ALS)
On-the-fly compartmentalisation
- Phase separation relies on a subtle interplay between entropy and enthalpy
- Slightly changing some conditions can suppress/enhance phase separation
- Condensates can quickly adapt to environmental changes!
A visual example
- We take a 5-component mixture $\to$ we need to set 15 different interactions
- For this particular choice the resulting system is homogeneous
- We change a single interaction $\to$ phase separation


LLPS in proteins
- Abnormal LLPS is connected to many diseases (Alberti and Dormann, Annu. Rev. Genet. 2019)
- Relative concentrations are deeply connected to the overall thermodynamic stability (Riback et al, Nature 2020)
- LLPS seems to be involved in transcriptional control (Hnisz et al, Cell 2017)
- Some types of condensates act as scaffolds that concentrate other (client) molecules (Banani et al, Cell 2016)
- The local mechanical properties affect the morphology and localisation of the condensates (Rosowski et al, Nat. Phys. 2020)
Some motivating problems
- The interior of the cell contains thousands of different macromolecules
- Evolutionary pressure optimises mutual interactions in the proteome
-
In vivo and in vitro experiments don't always match (Jain and Vale, Nature 2017)
Our idea$^\dagger$
Make an organism express artificial proteins designed to phase separate
The artificial proteins (expressed in a yeast strain through plasmids) should
- be extraneous to the cell
- bond through a lock-and-key mechanism
- be multivalent (e.g. can interact with multiple partners)
The goal: build a synthetic toolkit to study LLPS in vivo
$^\dagger$ Emmanuel Levy's ingenious idea, to be really honest
The building blocks

- Two components (A and B) that can form up to 4 and 2 bonds, respectively
- Their size is chosen so that multiple bonding is unlikely (if not impossible)
- The lock-and-key attraction strength can be tuned with point mutations
A closer look

A preliminary check
Control sample: no phase separation if the lock-and-key attraction is disabled
Lock-and-key enabled: the components co-localise and phase separate!
Quantifying the phase behaviour
We draw a negative of the low-$\rho$ part of the phase diagram!
N.B.: the high-concentration part of the phase diagram cannot be accessed
A simple (but not too simple) model
We retain the main ingredients and no more
- Multivalency (a.k.a. limited valence in the colloidal field)
- Single-bond-per-site
- A lock-and-key mechanism (a.k.a. bond specificity)
The base model we choose is a patchy particle
Bianchi et al., Phys. Chem. Chem. Phys. 2017
The theory
The free energy of a self-assembling system can be written as
$$
\beta f = \beta f_{\rm ref} + \beta f_{\rm bond}
$$
- $\beta f_{\rm bond}$ is the contribution due to bonding
- $\beta f_{\rm ref} = \beta f_{\rm id} + x \ln (x) + (1 - x) \ln(1-x) + \beta f_{\rm ex}$
- At low density $\beta f_{\rm ex} \approx \rho \left(x^2 B_2^{AA} + 2x(1 - x) B_2^{AB} + (1-x)^2 B_2^{BB} \right)$, where
- $x$ is the composition
- $B_2^{AA}$, $B_2^{AB}$, $B_2^{BB}$ are the second virial coefficients
Wertheim, J. Stat. Phys.
1984, Bianchi, et al, Phys. Rev. Lett.
2006
Connecting theory and simulations
-
The second virial coefficients can be estimated as
$$
B_{ij} = -\frac{1}{2} \int_0^\infty 4 \pi r^2 \left( e^{-\beta V_{ij}(r)} - 1\right) dr
$$
where $V_{ij}(r)$ is the reference interaction potential between species $i$ and $j$
-
$\beta f_{\rm bond}$ is linked to the free energy cost of a single bond
Both can be computed with two-body simulations $\to$ phase diagram
The coarse-graining strategy

Choosing the model
Small patches $\to$ single-bond-per-patch but slow equilibration

Big patches $\to$ easier to find partners but multiple bonding possible

The best of both worlds
Introducing the bond-swapping mechanism
Configuration |
 |
 |
 |
V2 |
$-\epsilon$ |
$-2\epsilon$ |
$-\epsilon$ |
V3 |
$0$ |
$\lambda\epsilon$ |
$0$ |
The value of $\lambda$ controls the behaviour
- $\lambda \geq 1$ $\to$ single-bond-per-patch
- $\lambda = 1$ $\to$ free swapping!
F. Sciortino, Eur. Phys. J. E (2017), L. Rovigatti et al., Macromolecules (2018)
Bond swapping $\to$ no arrest
Systems can be equilibrated down to $T \to 0$

L. Rovigatti et al., Macromolecules (2018)
Free lunch, for once
Faster equilibration for $\lambda = 1$

Numerical details
- Binary mixture of divalent and tetravalent particles, varying $\rho$, $x$, $T$ ($\propto$ affinity$^{-1}$)
- Sedimentation simulations for the phase diagrams in and out of equilibrium
- Constant-volume simulations to compare to dynamic data
- Explore how changing the model affects the thermodynamics


Sedimentation
We fit the equation of state to extract the coexisting $\rho_2$ and $\rho_4$

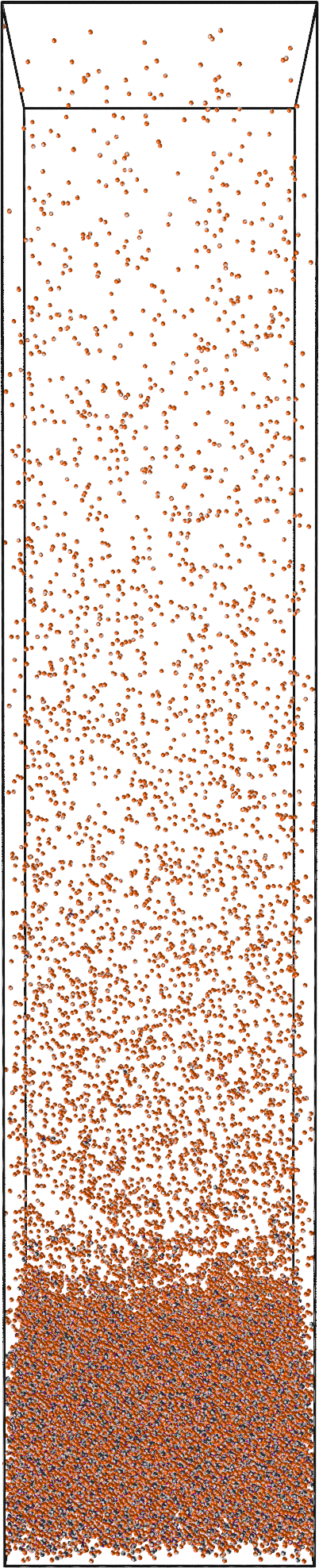
Experimental phase diagrams


- The coexistence region is $\approx$ symmetric with respect to stoichiometry conditions
- It enlarges as affinity (i.e. bond strength) increases
- Something happens at high affinities: out-of-equilibrium effects?
Theoretical phase diagrams

- The coexistence region is centred on the perfect stoichiometry line
- It enlarges as affinity (
i.e.
bond strength) increases
We reproduce all the equilibrium qualitative trends
Numerical phase diagrams
Numerical phase diagrams in and out of equilibrium


We see the same qualitative shift measured in experiments
Out-of-equilibrium effects!


- Fluorescence Recovery After Photobleach (FRAP) data on single cells
- Large spread between the curves, especially at low affinity
- At large affinities there is $\approx$ no recovery after 25 seconds
Model extensions
We can build on the model to assess the role
- of non-specific attractions
- of defects (example: some tetramers can form only 3 bonds)
- of the flexibility/geometrical arrangements of the patches



Conclusions
- We can engineer synthetic biomolecular condensates with tunable properties
- The phase behaviour of the system can be directly measured
- Notwithstanding the complexity of the cell environment, experiments agree with coarse-grained simulations/theory, in and out of equilibrium
- The system can be used to test hypothesis and develop new methods (see e.g. Mc Laughin et al, Mol. Biol. Cell (2020))
References
- Bond-swap in patchy systems: L. Rovigatti et al., Macromolecules 51, 1232 (2018)
- Yeast system: M. Heidenreich et al., Nat. Chem. Biol. 16, 939 (2020)
Acknowledgements
Work done in collaboration with:
- E. Locatelli (University of Vienna)
- J. P. K. Doye (University of Oxford)
- S. K. Nandi (Tata Institute of Fundamental Research)
- M. Heidenreich (Weizmann Institute)
- S. A. Safran (Weizmann Institute)
- E. D. Levy (Weizmann Institute)
The building blocks

What is the influence of $\lambda$?
Systems with $\lambda = 1$ and $10$ have the same phase diagrams

Direct coexistence
The recipe
- Equilibrate at small pressure (the specific value is not important)
- Enlarge the box along one direction (here $z$)
- Compute the density profile and extract the coexisting densities

Sedimentation vs. direct coexistence
$x = \frac{\rho_4}{\rho_2 + \rho_4}$, $\rho = \rho_2 + \rho_4$
Less numerically demanding but no out-of-equilibrium options
Equilibrium phase diagrams
The phase diagram enlarges with the bond strength (affinity), as in the experiments

FRAP pictures

Simple models $\to$ complex behaviour
Limited valence
E. Bianchi, J. Largo, P. Tartaglia, E. Zaccarelli and F. Sciortino, Phys. Rev. Lett. (2006)
Simple models $\to$ complex behaviour
Self-assembly vs. phase separation


F. Sciortino, A. Giacometti and G. Pastore, Phys. Rev. Lett. (2009)
J. Russo, J. M. Tavares, P. I. C. Teixeira, M. M. Telo da Gama and F. Sciortino, Phys. Rev. Lett. (2011)
L. Rovigatti, J. M. Tavares and F. Sciortino, Phys. Rev. Lett. (2013)